Ultracold Rb-KRb Collisions Reveal Energy Transfer
In the intricate world of ultracold chemistry, a new frontier has been crossed: directly observing energy transfer from atomic hyperfine states to molecular rotational states during collisions involving atoms and molecules. While energy exchange among mechanical degrees of freedom, such as translation and rotation, has been extensively studied and relatively well understood, the coupling involving […]

In the intricate world of ultracold chemistry, a new frontier has been crossed: directly observing energy transfer from atomic hyperfine states to molecular rotational states during collisions involving atoms and molecules. While energy exchange among mechanical degrees of freedom, such as translation and rotation, has been extensively studied and relatively well understood, the coupling involving spin degrees of freedom has remained largely enigmatic. The recent groundbreaking study by Liu et al. sheds unprecedented light on this phenomenon, unveiling the complex interplay between atomic spins and molecular rotation in a prototypical ultracold atom–molecule collision system involving rubidium (Rb) atoms and potassium-rubidium (KRb) molecules.
At temperatures approaching absolute zero, where quantum effects reign supreme, the researchers focused on collisions between ^87Rb atoms initially prepared in the hyperfine state (|Fa, M{F_a}\rangle = |2, 2\rangle) and ^40K^87Rb molecules in their electronic ground state X ^1Σ^+ with rotational quantum number (N = 0). By probing these collisions, the team observed real-time, state-to-state transitions whereby the Rb atom’s hyperfine state relaxes to (|1, 1\rangle), simultaneously transferring its hyperfine energy to the rotational motion of the KRb molecule, which exhibited final rotational states (N = 0, 1,) or (2).
This direct observation marks a significant advance because it illustrates an explicit pathway through which spin angular momentum can convert into molecular mechanical rotation—a coupling mechanism that until now had largely eluded experimental confirmation. The hyperfine interaction, arising from couplings between the nuclear spin and electronic magnetic moments inside the atom, typically dominates a system’s internal energy landscape, whereas molecular rotation reflects the classical mechanical angular momentum associated with nuclei in the molecule. How these fundamentally different angular momenta convert and distribute energy in ultracold collision processes is vital for understanding and controlling chemical reactivity in the quantum regime.
To complement their high-resolution spectroscopic measurements, the authors performed comprehensive quantum scattering calculations. These calculations rigorously incorporated the complex coupling between spin and rotational degrees of freedom within the collision complex, although under the simplifying assumption that the KRb molecule behaves as a rigid rotor moving along a single, well-defined potential energy surface (PES). This approach permitted detailed theoretical predictions of the end-state rotational distributions of KRb molecules post-collision.
Interestingly, the theoretical results did not fully match the experimental observations, even after extensive tuning of the atom–molecule interaction potential to achieve optimal agreement. The discrepancies highlighted limitations in the rigid-rotor and single-surface approximation commonly employed in ultracold collision models. Such assumptions omit the involvement of vibrational motion and possible non-adiabatic effects due to coupled electronic states, features increasingly recognized as critical in low-temperature reaction dynamics.
Significantly, the team’s ab initio electronic structure calculations revealed the presence of a conical intersection, an intersection of two potential energy surfaces, which lies energetically accessible at short interparticle distances within the collision complex. Conical intersections are well-known in molecular photochemistry for facilitating rapid and efficient non-radiative transitions between electronic states, acting essentially as “funnels” for energy redistribution. Their presence in ultracold atom–molecule collisions implies that vibrational degrees of freedom and electronic non-adiabatic coupling cannot be neglected when describing hyperfine to rotational energy transfer.
This insight reshapes the theoretical landscape of ultracold collision physics by acknowledging and demonstrating that spin–rotation interactions are not just a perturbative effect but instead emerge significantly from the interplay of multiple intertwined degrees of freedom—spin, rotation, vibration, and electronic state coupling—at short range. It challenges existing theoretical frameworks to incorporate more sophisticated multi-surface, non-adiabatic models that fully capture the complexity revealed by these precise experiments.
From an experimental standpoint, the researchers employed cutting-edge ultracold molecule preparation and control techniques, enabling exquisite state selectivity. The ability to prepare both atomic and molecular species in well-defined hyperfine and rotational states allowed for direct tracking of energy transfer pathways. Such precise control is essential for teasing out subtle spin-related energy exchanges, which might otherwise be obscured within ensemble-averaged measurements.
The findings have profound implications for future endeavors in quantum-controlled chemistry and quantum information science. Hyperfine and rotational states serve as promising candidates for qubits and quantum memories, especially in systems where spin–rotation coupling can be harnessed to mediate interactions or to transfer coherence between different physical degrees of freedom. Understanding and controlling such couplings at the fundamental level open avenues for engineering hybrid quantum devices combining atoms and molecules.
Furthermore, the study establishes a rigorous benchmark for future theoretical efforts aiming to accurately model ultracold chemical reactions involving spins. The observed deviations from current theoretical models highlight the necessity to move beyond single-potential, rigid-rotor approximations, and to incorporate fully coupled multi-dimensional interactions including vibrational motion and conical intersections. Such developments are expected to refine predictions of reaction rates, product distributions, and coherence properties, which are crucial for designing experiments and applications in cold chemistry.
In the context of fundamental physics, this research offers a rare view into angular momentum coupling mechanisms that bridge electronic, nuclear, and rotational degrees of freedom, deepening our understanding of how complex quantum systems redistribute energy. It intersects with broader themes such as spin-orbit coupling, collision-induced spin relaxation, and the role of electronic structure in dictating dynamical outcomes—areas that continue to energize atomic, molecular, and optical physics.
Moreover, by directly observing hyperfine-to-rotational energy transfer at ultracold temperatures, the study reflects the increasing capability of experimentalists to probe and manipulate quantum systems at an unprecedented level of detail. This precision not only aids in validating fundamental quantum scattering theories but also sets the stage for exploring more complex systems, such as polyatomic molecules or spin networks, where rich dynamics governed by spin-mechanical couplings may be harnessed.
Looking ahead, the research invites further inquiry into mechanisms by which conical intersections enhance spin–rotation coupling, and how vibrational excitations contribute to energy redistribution pathways. It points to the promise of integrating vibrational degrees of freedom explicitly in future theoretical models and experimental probes, potentially through the use of spectroscopic techniques sensitive to vibrational state populations.
Equally exciting is the prospect of applying these insights to control chemical reactivity by manipulating hyperfine states or coherently steering collisional outcomes via tailored external fields. Such control protocols would bring quantum-state-resolved chemistry from fundamental physics laboratories closer to practical applications in precision measurement, quantum simulation, and even catalysis at the quantum scale.
In conclusion, the landmark study by Liu, Zhu, Luke, and colleagues reveals the subtle yet significant bridges connecting atomic spins and molecular rotation under ultracold conditions, corroborating some theoretical expectations while exposing gaps that will fuel future research. It stands as a testament to the progress at the crossroads of quantum control, molecular physics, and chemical dynamics, charting a roadmap toward a deeper understanding of quantum matter and its applications in emerging quantum technologies.
Subject of Research: Hyperfine-to-rotational energy transfer in ultracold atom–molecule collisions
Article Title: Hyperfine-to-rotational energy transfer in ultracold atom–molecule collisions of Rb and KRb
Article References:
Liu, YX., Zhu, L., Luke, J. et al. Hyperfine-to-rotational energy transfer in ultracold atom–molecule collisions of Rb and KRb. Nat. Chem. (2025). https://doi.org/10.1038/s41557-025-01778-z
Image Credits: AI Generated
Tags: advancements in ultracold collision studiesatomic spin and energy couplingenergy transfer in atomic collisionsexperimental observations in quantum physicshyperfine states in ultracold atomsmolecular rotational statesquantum effects at absolute zeroreal-time state transitionsrotational quantum numbers in moleculesrubidium and KRb interactionsultracold atom-molecule systemsultracold chemistry
What's Your Reaction?
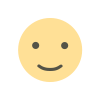
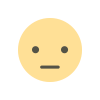
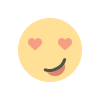
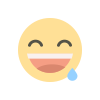
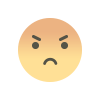
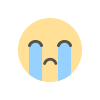
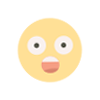