Metabolic Pathway Control via Cellular Biomolecular Condensates
In the intricate world of cellular biology, the regulation of metabolism often revolves around complex networks of interactions and spatial organization. Recent advances have illuminated a striking phenomenon that cells harness to fine-tune these processes: phase separation of biomolecules. This fundamental mechanism gives rise to membraneless compartments known as biomolecular condensates, structures that have captivated […]

In the intricate world of cellular biology, the regulation of metabolism often revolves around complex networks of interactions and spatial organization. Recent advances have illuminated a striking phenomenon that cells harness to fine-tune these processes: phase separation of biomolecules. This fundamental mechanism gives rise to membraneless compartments known as biomolecular condensates, structures that have captivated scientists with their ability to concentrate or exclude specific macromolecules. A newly published study now delves deep into how these condensates can be strategically leveraged to control metabolic pathways, enhancing both yield and selectivity in ways previously unappreciated.
Biomolecular condensates represent a paradigm shift in cellular compartmentalization. Unlike traditional organelles enclosed by membranes, these dynamic condensates form through liquid-liquid phase separation, a process where biomolecules like proteins and nucleic acids spontaneously demix from their surroundings to create droplet-like domains. This separation is not merely spatial but functional, creating microenvironments that can dramatically alter biochemical reactions. The condensates’ capability to enrich enzymes or substrates selectively reshapes classical views of metabolic control, suggesting that cells exploit these structures for precise pathway regulation.
The research spearheaded by Lee, Walls, Siu, and colleagues, soon to be featured in Nature Chemical Engineering, provides a compelling theoretical and experimental framework to quantify how condensates influence metabolic output. Their findings indicate that the success of condensate-mediated pathway control can be distilled into a single predictive metric—a blend of two key parameters: the fraction of enzyme molecules that partition into the condensate and the relative change in enzyme activity inside these compartments compared to the cytosol. This dual-parameter model offers unprecedented simplicity in predicting pathway outcomes influenced by condensate formation.
Enzymes embedded within biomolecular condensates do not behave identically to their free-floating counterparts. Partitioning refers to the preferential localization of enzymes within condensates, which depends on molecular interactions defining the condensate’s composition and physical chemistry. This sequestration can significantly alter local enzyme concentration, enhancing catalytic efficiencies through proximity effects and substrate channeling. Simultaneously, the biochemical environment inside the condensate may modulate enzymatic activity—either boosting or diminishing it—due to altered crowding, pH, ionic strength, or cofactor availability. Together, these factors influence the net metabolic flux and product formation.
Critically, the team demonstrated robustness of their predictive model by engineering synthetic biomolecular condensates within yeast cells. By utilizing genetically encoded condensate-forming domains tethered to metabolic enzymes, they effectively rewired acetoin biosynthesis, a metabolic pathway with industrial and biotechnological relevance. Their experiments validated that enzymes selectively sequestered in condensates showed altered catalytic profiles, aligning closely with the proposed metric’s predictions. This synthetic biology approach not only confirms theoretical principles but also opens avenues for practical applications in metabolic engineering.
These findings touch upon several long-standing questions in cell biology and bioengineering. While natural biomolecular condensates such as P-bodies, stress granules, and nucleoli have been studied extensively, their direct influence on metabolic pathways has remained relatively elusive. By providing a quantitative handle, this study bridges a critical knowledge gap. The approach elucidates how cells might exploit phase separation to tune metabolism in response to environmental stimuli—rapidly modulating flux without transcriptional or translational remodeling.
Furthermore, this work carries significant implications for metabolic engineering of microbial and mammalian cells. Traditional strategies focus on genetic or enzymatic alterations aimed at manipulating pathway enzymes directly. Incorporating phase separation as a design principle enables a complementary strategy: engineering the spatial distribution and microenvironment of enzymes. This spatial control could unlock new levels of precision in optimizing flux, yield, and product specificity across diverse biochemical applications such as biofuel production, pharmaceuticals, and synthetic biology circuits.
From a chemical engineering perspective, the study offers a fresh perspective on reaction compartmentalization. The condensate-based model redefines how reactors might be miniaturized intracellularly, where reaction rates can be enhanced not only by increasing enzyme concentration but also by carefully modulating enzyme activity through microenvironment properties. This insight lays the groundwork for next-generation bioreactors and manufacturing platforms that leverage intracellular crowding and phase behavior to push biological production boundaries.
Delving into the technical details, the researchers employed a coarse-grained analytical framework to capture the essence of enzyme partitioning and activity modulation. By simplifying the complex interactions within condensates to measurable parameters, the model achieves a balance between theoretical rigor and experimental applicability. This allows researchers to prioritize quantifiable traits—such as enzyme affinity for condensate constituents and kinetic alterations within—that can be experimentally accessed through fluorescence imaging, activity assays, and microfluidic analysis.
Beyond enzymatic activity, the physicochemical properties of the condensate environment emerge as a critical determinant. Factors such as viscosity, diffusivity, and local crowding influence substrate availability and product removal—affecting turnover and pathway throughput. The study systematically integrates these effects into the overarching metric, highlighting the importance of considering both molecular and environmental dynamics in condensate biology.
The versatility of biomolecular condensates also presents intriguing opportunities for selective pathway control. Certain metabolic branches or competing reactions can be preferentially enhanced or suppressed by fractionally sequestering enzymes, thus allocating cellular resources more efficiently. This provides a mechanism for dynamic rewiring of metabolism, allowing cells or engineered systems to prioritize certain outputs based on internal or external demands, such as stress responses or nutrient availability.
Importantly, the experimental validation in yeast serves as an accessible model system illustrating condensate function in eukaryotic cellular contexts. The genetic tractability of yeast enables rapid iteration of condensate designs and pathway configurations, setting the stage for translating principles to higher organisms or industrial strains. The study’s engineered synthetic condensates demonstrate controllable, tunable formation and dissolution, underscoring the potential for real-time regulation of metabolism.
The broader implications touch on fundamental biological processes as well. Many diseases, including neurodegenerative disorders and cancers, have been linked to dysfunctional phase separation and condensate dysregulation. Understanding how condensates regulate core metabolic pathways offers insight into pathogenesis mechanisms and potential therapeutic targets. Furthermore, engineering condensates could be envisaged as a strategy to restore or manipulate cellular function in disease contexts.
As the field advances, quantification of the critical parameters identified in this work will become increasingly important. The authors advocate for the integration of advanced biophysical tools and high-throughput assays to systematically measure enzyme partition fractions and activity changes within diverse condensate types. Such detailed characterization will feed back into model refinement and predictive accuracy, propelling condensate-enabled metabolic engineering to new heights.
In conclusion, this landmark study reframes the cellular metabolic landscape by highlighting biomolecular condensates as a powerful modality for pathway control. Their dual-parameter predictive metric simplifies the complex interplay between enzyme localization and activity modulation within these membraneless microreactors. By bridging theory and synthetic biology, the research provides a blueprint not only for understanding native cellular metabolism but also for engineering sophisticated metabolic systems with unprecedented precision. As this frontier unfolds, leveraging phase separation promises to revolutionize how scientists harness and remodel biological chemistry across multiple domains.
Subject of Research: Regulation and optimization of metabolic pathways through biomolecular condensates formed by phase separation in cells.
Article Title: Principles of metabolic pathway control by biomolecular condensates in cells.
Article References:
Lee, D., Walls, M.T., Siu, K.H. et al. Principles of metabolic pathway control by biomolecular condensates in cells. Nat Chem Eng 2, 198–208 (2025). https://doi.org/10.1038/s44286-025-00193-y
Image Credits: AI Generated
DOI: https://doi.org/10.1038/s44286-025-00193-y
Tags: biochemical reaction microenvironmentsbiomolecular condensates and metabolismcellular compartmentalization mechanismsdynamic biomolecular structuresenzymatic pathway enhancementliquid-liquid phase separationmembraneless organelles in biologymetabolic pathway regulationNature Chemical Engineering research findingsphase separation in cellsquantitative analysis of metabolic controlspatial organization of biomolecules
What's Your Reaction?
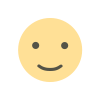
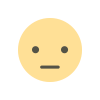
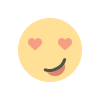
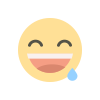
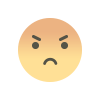
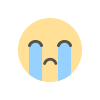
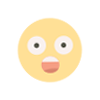