Scientists make and test efficient water-splitting catalyst predicted by theory
UPTON, N.Y. — Hydrogen (H2) is a promising fuel for reducing greenhouse gases, especially if produced by using renewable energy to split water molecules (H2O). But as simple as it may seem to break water into hydrogen and oxygen, the chemistry is complex. Two separate simultaneous electrochemical reactions each require catalysts, chemical “deal makers” that help […]
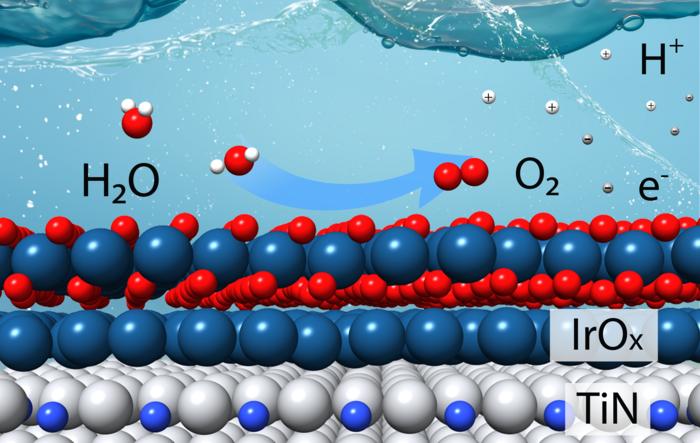
UPTON, N.Y. — Hydrogen (H2) is a promising fuel for reducing greenhouse gases, especially if produced by using renewable energy to split water molecules (H2O). But as simple as it may seem to break water into hydrogen and oxygen, the chemistry is complex. Two separate simultaneous electrochemical reactions each require catalysts, chemical “deal makers” that help break and remake chemical bonds. Now, scientists at the U.S. Department of Energy’s (DOE) Brookhaven National Laboratory and Columbia University say they’ve developed a new efficient catalyst for the more challenging part: the oxygen evolution reaction.
Credit: Tianyou Mou/Brookhaven National Laboratory
UPTON, N.Y. — Hydrogen (H2) is a promising fuel for reducing greenhouse gases, especially if produced by using renewable energy to split water molecules (H2O). But as simple as it may seem to break water into hydrogen and oxygen, the chemistry is complex. Two separate simultaneous electrochemical reactions each require catalysts, chemical “deal makers” that help break and remake chemical bonds. Now, scientists at the U.S. Department of Energy’s (DOE) Brookhaven National Laboratory and Columbia University say they’ve developed a new efficient catalyst for the more challenging part: the oxygen evolution reaction.
As described in a paper just published in the Journal of the American Chemical Society, the catalyst was designed “from the bottom up” based on theoretical calculations seeking to minimize the amount of iridium, an expensive metal used as a catalytic material, and to maximize the catalyst’s stability in acidic conditions. When the team created models of the catalyst and tested them in the lab, the results validated the predictions. Then, the scientists made a powder form of the catalyst, like those used in industrial applications, and showed it can efficiently produce hydrogen in a water-splitting electrolyzer.
“In this real-world test, our catalyst is about four times better than the state-of-the-art commercially available iridium catalyst,” said Jingguang Chen, a chemical engineer at Columbia University with a joint appointment in the Chemistry Division at Brookhaven Lab who led the research. In other words, the new catalyst requires four times less iridium to produce hydrogen at the same rate as the commercial variety — or produces hydrogen four times faster for the same amount of iridium.
Brookhaven Lab theoretical chemist Ping Liu, who led the calculations that underpin the catalyst’s design, said, “This study demonstrates how you can go from a theory-driven understanding of what’s happening at the atomic level to designing a catalyst for a practical use. Our work gives us a better understanding of how this catalyst works and gets us closer to the real-world application.”
The remaining challenge is to scale up production.
“We are only making milligrams of catalyst per batch,” Chen said. “If you want to make megatons of green hydrogen, you’d need kilograms or tons of catalyst. We can’t make this at that large scale yet.”
Reducing iridium
Iridium is the catalyst of choice for the oxygen evolution reaction, which takes place at the anode of an electrolyzer. It provides the electrically charged active sites that separate tightly bound hydrogen ions (H+) from oxygen (O). In addition to freeing the H+ ions — which contribute to the harshly acidic reaction conditions — the reaction produces oxygen gas (O2) and electrons. Those electrons are needed for the second, less challenging “hydrogen evolution” reaction: the pairing up of hydrogen ions to form hydrogen gas at the electrolyzer’s cathode.
“Iridium is currently one of the only stable elements for the oxygen evolution reaction in acid,” Chen said. That’s “unfortunate,” he noted, because “iridium is even more rare, and more expensive, than platinum.”
Hence, the motivation for reducing the amount of iridium.
“In industrial catalysts made of nanoscale particles, only atoms on the surface participate in the reaction,” Chen said. “That means most of the iridium on the inside of the particle is wasted.”
Maybe instead of using a particle that is all iridium, a catalyst could be made of a less-expensive material with iridium only on the surface, the team reasoned.
With funding from a DOE initiative to advance clean-energy technologies, they had been exploring the use of earth-abundant elements such as titanium. They found that combining titanium with nitrogen provided enough stability for these “titanium nitrides” to survive acidic reaction conditions. Perhaps titanium nitride could serve as the core of iridium-coated catalytic particles.
But how much iridium should be layered on top? This is where the theoretical calculations come in.
Calculating an ideal structure
“We used ‘density functional theory’ calculations to model how different overlayers of iridium on titanium nitride would affect the stability and activity of the catalyst under acidic oxygen evolution reaction conditions,” said Liu. She and her team used computing resources at Brookhaven Lab’s Center for Functional Nanomaterials (CFN) and at the National Energy Research Scientific Computing Center (NERSC) at DOE’s Lawrence Berkeley National Laboratory to run the simulations.
The calculations predicted that one layer of iridium would not be sufficient to drive the oxygen evolution reaction but that two or three layers would improve both performance and catalytic stability.
“These were sort of pre-screening experiments,” Liu said. “Then, we turned these screening results over to the experimental team to make real catalysts and evaluate their catalytic activity.”
Validating the predictions
First, the team created thin films in which they could create carefully controlled layers that closely resembled the surfaces used in the theoretical modeling calculations. They also created powdered samples composed of small nanoscale particles, the form the catalyst would take in industrial applications. Then, they studied the thin films — including the interfaces between the layers — and the nanoparticles using a variety of techniques.
These included transmission electron microscopy at CFN and X-ray spectroscopy studies at the Quick X-ray Absorption and Scattering (QAS) beamline of the National Synchrotron Light Source II (NSLS-II), a source of bright X-rays for deciphering samples’ chemical and physical properties.
“Our hypothesis was that if the iridium bonds to the titanium nitride, this bonding would stabilize the iridium and improve the reaction,” Chen said.
The characterization studies bore out the predictions.
“The synchrotron studies revealed the oxidation states and local coordination environment of the iridium and titanium atoms under reaction conditions,” Chen said. “They confirmed that the iridium and titanium are interacting strongly.”
“Mapping the elements of the nanoparticles at CFN confirmed the particle sizes and compositions, including the presence of iridium oxides on the surface over titanium nitride supports,” he added.
Liu emphasized that the characterization studies informed the scientists’ understanding of the catalyst.
“We found that the interaction between iridium and titanium is not only helpful to the stability of the catalyst but also in fine tuning its activity,” she said. “The charges change the chemistry in a way that improves the reaction.”
Specifically, charges transferred from titanium to the iridium surface alter the electronic structure of the iridium active sites to optimize the binding of reaction intermediates, she explained.
“Going from one to three layers of iridium, you increase the charge transfer from the nitride to the top iridium significantly,” Liu noted. But the difference between two and three layers was not very large. Two layers might be enough to allow high stability, activity, and low cost.
To make this catalyst ready for real-world use, the scientists pointed out that, in addition to tackling the challenge of scaling up production, there could also be improvements to optimize consistency of the powders.
“When we make thin films, we can control the layers, but with powder synthesis, we don’t have that kind of control,” Chen said. “Our powder particles don’t have a continuous iridium shell around them. But this study provides guidelines industrial chemists could use to make true core-shell structures with a uniform thin layer of iridium,” he said.
Such catalysts could help lower the cost of water splitting and bring scientists closer to producing large quantities of green hydrogen.
This work was funded by the DOE Office of Science. CFN, NSLS-II, and NERSC all operate as DOE Office of Science user facilities.
Brookhaven National Laboratory is supported by the Office of Science of the U.S. Department of Energy. The Office of Science is the single largest supporter of basic research in the physical sciences in the United States and is working to address some of the most pressing challenges of our time. For more information, visit science.energy.gov.
Follow @BrookhavenLab on social media. Find us on Instagram, LinkedIn, X, and Facebook.
Journal
Journal of the American Chemical Society
DOI
10.1021/jacs.4c02936
Article Title
Theoretical Prediction and Experimental Verification of IrOx Supported on Titanium Nitride for Acidic Oxygen Evolution Reaction
Article Publication Date
10-Jun-2024
What's Your Reaction?
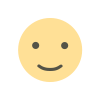
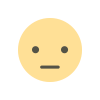
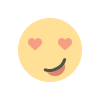
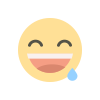
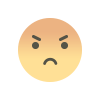
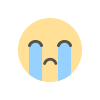
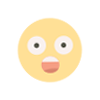