Mini-Organs in a Dish to Revolutionize Rare Disease Therapy
Children born with rare genetic disorders often encounter a seemingly insurmountable succession of health challenges, each rooted in a missing or malfunctioning protein caused by an underlying mutation. The quest to treat these conditions has intensified over the past decade, as the scientific community has come to recognize that classical pharmacological approaches may not suffice […]

Children born with rare genetic disorders often encounter a seemingly insurmountable succession of health challenges, each rooted in a missing or malfunctioning protein caused by an underlying mutation. The quest to treat these conditions has intensified over the past decade, as the scientific community has come to recognize that classical pharmacological approaches may not suffice when the underlying problem resides deep within an individual’s genetic code. Researchers have therefore turned to innovative RNA therapeutics, notably antisense oligonucleotides (ASOs), to correct or circumvent these molecular glitches at their source. Yet, despite the excitement surrounding these custom-made RNA or DNA fragments, a major bottleneck has persisted. Traditional approaches to designing, validating, and optimizing ASOs—particularly for unique, patient-specific mutations—can be extraordinarily slow and expensive. This logistical hurdle has meant that for many children in dire need of targeted therapies, a working treatment remains heartbreakingly out of reach. Now, in a study that signals a landmark shift in how we might accelerate drug development for rare genetic conditions, Means et al. have devised a robust and scalable system built on the power of induced pluripotent stem cells (iPS cells). Harnessing these cells, the researchers can assemble organ-like tissues, called organoids, that recapitulate key functional attributes of the human heart, brain, or skeletal muscle. These living three-dimensional “mini-organs” present an unprecedented platform for testing the impact of ASOs on mutated genes, thus paving the way for swift, personalized solutions.
The impetus for developing such a system stems from the pressing need to adapt treatments to each individual’s genetic variation. Historically, certain ASOs have been crafted to skip problematic exons in genes tied to severe disorders such as Duchenne muscular dystrophy, which stems from mutations in the dystrophin gene. The principle is elegant: by adding a small piece of synthetic RNA that instructs cellular machinery to ignore a specific, mutation-ridden exon, the cell can then stitch together the rest of the protein transcript. While the resultant dystrophin may be shorter than normal, it often remains functional enough to preserve muscle integrity. Several of these ASOs have already garnered regulatory approval for human use. Yet, each patient may carry a slightly different mutation, necessitating a distinct “exon skip” or molecular strategy. Traditional research pipelines—culturing cells, verifying corrected protein production in test tubes, moving to animal models—can run for months or years. This timeline is untenable for children whose disease progresses rapidly, or for those who do not fit neatly into existing ASO designs. Means et al. recognized that cell-based models, if universally accessible and seamlessly scalable, could drastically speed up the process of matching patients with effective, custom-made RNA interventions.
In their recent publication, the authors describe their integrated approach, starting from a large-scale reprogramming pipeline that transforms standard patient blood samples into iPS cells. Conventional methods to derive iPS cells are often laborious, requiring extensive hands-on time, specialized growth media, and highly controlled lab environments. Moreover, creating iPS cells from multiple patients in parallel can be logistically daunting, with each cell line requiring unique sets of reagents, repeated validations, and round-the-clock monitoring. However, the new protocol consolidates all these steps into a streamlined process. Within two to three weeks, blood cells from up to a dozen different individuals can be simultaneously coaxed back to an embryonic-like, pluripotent state, then expanded in sufficient quantities to fill entire cryogenic storage libraries. The authors report that over a span of six months, they derived and banked nearly 300 iPS lines from a wide variety of patients, each line representing a distinct genetic background. Such reproducibility lays the foundation for exploring genetic diseases at scale, with each iPS cell line capturing the unique genome of an individual child.
From these iPS cells, the group then set about constructing three key tissue models: skeletal muscle cells, brain organoids, and heart organoids. Though organoids derived from pluripotent stem cells have captivated researchers for over a decade, their complexities are formidable. It is not enough to simply supply iPS cells with generic growth signals; one must carefully orchestrate each step of development—mimicking the precise cues that direct cells to form branching neural tubes or contractile cardiac tissues in vivo. Means et al. advanced these protocols in an accessible, cost-effective manner. Remarkably, within a matter of weeks, they obtained small but functional “mini-hearts” (cardiac organoids) that mimicked the rhythmic beating of actual heart tissue. Similarly, by exposing the iPS cells to specific differentiation factors, they generated brain organoids that show the early architecture of developing neural tissues. In parallel, they guided iPS cells down the path of the myogenic lineage to produce skeletal muscle cells. Each tissue type, once formed, recapitulated signature features of adult organ function—for instance, the organoids’ muscle cells contract in a coordinated pattern reminiscent of the beating heart.
Such replication of complex biology in a dish opens the door to a battery of functional tests. In healthy organoids, the structural protein dystrophin is robustly expressed in skeletal muscle and heart tissues. Meanwhile, an individual with Duchenne muscular dystrophy typically harbors a frameshift mutation that halts the production of dystrophin altogether, leading to fragile and easily damaged muscle cells. To explore whether their platform could genuinely test the efficacy of FDA-approved ASOs, the team employed iPS cells from a patient with the classical dystrophin mutation. They cultivated both heart organoids and skeletal muscle cells, confirming that these miniature tissues displayed negligible dystrophin levels and manifested weak, erratic contractions. Next, they administered an ASO clinically indicated for that particular exon skipping. Over the ensuing days, the researchers observed that the mini-hearts began contracting with a renewed vigor that matched or closely approximated organoids derived from healthy donors. Analysis of protein extracts confirmed that the treated tissues had begun producing a partially truncated, yet functional form of dystrophin. This reintroduction of dystrophin was enough to restore near-normal muscle contraction patterns, demonstrating that the tissue model faithfully mirrored the in vivo pathology—and, more importantly, that the ASO therapy was working at a cellular level. Subsequent tests with alternative ASOs aimed at different exons showcased similarly promising outcomes, reinforcing that this platform could handle a diverse range of patient mutations.
Such empirical demonstration cements the idea that a single set of protocols can handle an array of individualized ASOs, effectively bridging the gulf between academic research and personalized therapy. Instead of evaluating each prospective ASO in distinct animal models—a process that can be hampered by differences in species biology—clinicians and scientists can now see how human-derived tissues respond to treatments in a controlled, in vitro environment. This strategy offers deeper mechanistic insights, too. By analyzing gene and protein expression levels directly in the organoids, investigators can detect subtle changes in splicing patterns, gauge off-target effects, and confirm whether the reintroduced protein is properly localized. The system might thus serve as an early warning if a prospective ASO inadvertently disrupts other vital transcripts, or if the muscle fibers do not properly incorporate the new dystrophin. In short, rather than waiting for ambiguous, late-stage readouts in animal models or small human trials, one can refine and optimize promising therapies at the earliest possible juncture.
Still, the authors are forthright about the platform’s constraints. Although organoids display remarkable parallels to living tissues, they cannot perfectly emulate a fully mature organ. The immature state of the cells may mask issues that would surface in adult contexts, such as more complex immune interactions or changes in gene regulation that occur over years of development. In addition, these organoids represent only a handful of possible tissues—skeletal muscle, brain, and heart. Many diseases target entirely different organs: the liver, pancreas, or kidneys. While the protocols to create these additional organoids do exist, they often require specialized mediums, 3D scaffolding, or advanced bioreactor systems. Scaling those methods to the point that they can be seamlessly integrated into the present pipeline will take further ingenuity and technical expertise. Once accomplished, the expanded library of organoids could serve as a veritable compendium of patient-based disease models, pushing the boundary of how thoroughly we can test and refine RNA therapies.
Another factor is that certain mutations are so profoundly damaging that they might hinder normal development of the iPS-derived cells, potentially leading to inconsistent or incomplete organoid formation. In such cases, the question arises: how reliably can researchers interpret the therapeutic potential of an ASO if the underlying cells refuse to mature into the relevant tissues? This challenge emphasizes the importance of controlling genetic backgrounds. While the team currently obtains iPS lines from patients with widely diverse genetic profiles, a refined approach would leverage “isogenic controls.” That is, the same cell line can be genetically corrected at the targeted locus, allowing researchers to compare the corrected version directly to the original line. Alternatively, CRISPR-based methods could systematically introduce or repair specific mutations, thereby isolating the effect of each genetic alteration within the same baseline genome. Such comparative experiments would clarify whether an organoid’s abnormal phenotype is indeed the direct result of a single splicing defect or if it stems from background variants that are often present in real-world patients.
Variability across different batches of organoids remains another concern. Recreating identical mini-organs from the same patient line in distinct labs, or sometimes even at different times in the same lab, can be fraught with subtle changes in culture conditions—ranging from lot-to-lot variability in growth media to small fluctuations in temperature or pH. This can lead to discrepancies in organoid structure, viability, or functional readouts, making cross-experimental comparisons challenging. Means et al. address reproducibility by employing standardized protocols and carefully tracking each step of the differentiation process. Still, as other researchers adopt the pipeline, the field will likely converge on best practices for organoid generation, along with robust quality metrics that define exactly how an organoid’s “health” or maturity is measured. Such a consensus would expedite the broader deployment of these personalized models in labs worldwide.
Beyond these technical aspects, one must also consider the practical dimension: how do we convert the success of an in vitro organoid-based test into a clinically actionable therapy? In the case of Duchenne muscular dystrophy, approved ASOs already exist, but their clinical efficacy in halting disease progression remains partial at best. Delivery poses a stubborn challenge—achieving consistent distribution of ASOs throughout large muscle groups or across the blood–brain barrier can be difficult. Even if a mini-heart or mini-brain in a dish responds optimally to a given ASO, real human tissues could face obstacles related to blood supply, immune responses, or synergy with existing medications. Further research on targeted delivery vehicles, such as lipid nanoparticles or viral vectors, might integrate seamlessly with the organoid pipeline. Indeed, the pipeline could itself incorporate steps where experimental delivery systems are tested on organoids, evaluating not just the direct effect of the ASO but also whether it can penetrate deeper layers of cells or maintain stable expression over time.
Nevertheless, by substantially shortening the path from genotype to therapy evaluation, Means et al. have placed the realm of truly personalized medicine within closer reach. Suppose a child arrives at a genetic clinic with a newly characterized mutation in the dystrophin gene. Clinicians may send a blood sample for genomic sequencing and simultaneously launch the pipeline to convert those cells into iPS lines. Within a month or two, that child’s miniature muscles, hearts, or brain-like organoids could be grown. Scientists could then test multiple candidate ASOs, each designed to skip a relevant exon or correct the splicing error. Which agent triggers the best restoration of dystrophin? Which yields the fewest off-target consequences? The answers would emerge quickly, enabling a more confident path toward compassionate use or rapid trial enrollment—especially pressing for degenerative conditions that show early onset.
Equally promising is the pipeline’s potential utility beyond just muscular dystrophy. The principle of splicing-based interventions extends to a wide array of other disorders. Rare diseases resulting from splicing anomalies in CFTR (the cystic fibrosis transmembrane conductance regulator) or in genes underlying certain neurological conditions may all benefit from carefully crafted ASOs. Similarly, central nervous system disorders that hinge on a faulty transcript might be amenable to correction if the splicing machinery can be modulated in a targeted way. Integrating these additional disease models would require refining the organoid platform to recapitulate advanced lung or neuronal tissue, but the blueprint is there. Each success story in the pipeline paves the way to replicate or expand methods for a more diverse set of conditions.
Beyond the urgent demands of rare disease, these revelations also carry broad implications for how we conceptualize preclinical modeling. Traditional cell monolayers in a Petri dish, while still invaluable for certain assays, often fail to replicate the intricate architecture and mechanical signals of an actual tissue. Meanwhile, genetically modified animal models might not recapitulate the same splicing patterns or gene expression relevant to humans. Organoids offer an attractive middle ground, providing three-dimensional structure with human-specific biology, but they remain more straightforward to manipulate than a full living organism. By systematically applying organoids to test RNA therapies, the research community may unearth novel insights about how splicing is regulated in development, or how certain cell types respond to partial correction of a mutated gene.
Means et al. have presented a well-rounded approach to the next generation of personalized medicine, rooted in an accessible pipeline to create iPS cells from patient blood, differentiate them into relevant organoids, and administer custom RNA therapies. While challenges remain—immature organoid phenotypes, variations in batch quality, and difficulties translating to in vivo therapy—the potential to rapidly iterate and refine interventions is undeniable. This synergy between advanced stem-cell technology and molecular medicine could transform how we approach genetic disorders, particularly those that strike early in life and progress rapidly. The transition from bench to bedside may still demand further studies, but the conceptual leap has been made. A future in which each child with a devastating genetic condition can have a tailored splicing correction therapy, validated in their own tissue analogs before it even enters their bloodstream, no longer reads like science fiction. Rather, it stands on the horizon, beckoning us to continue refining and scaling up these extraordinary breakthroughs so that any child, no matter how rare or complex their mutation, can hope for a targeted and effective treatment.
Subject of Research: Development of a scalable platform using patient-derived mini-organs (organoids) for personalized RNA therapy testing
Article Title : A scalable system using mini-organs to test personalized RNA therapy
News Publication Date : 22 January 2025
Article Doi References : https://doi.org/10.1038/d41586-025-00078-3
Image Credits : Scienmag
Keywords : iPS cells, Organoids, Antisense oligonucleotides, Duchenne muscular dystrophy, Gene therapy, Personalized medicine, Stem-cell differentiation, Frameshift mutation
Tags: accelerating drug development for rare diseasesadvancements in therapeutic strategies for childrenantisense oligonucleotides in medicineantisense oligonucleotides in treatmentbreakthroughs in rare genetic disorder therapieschallenges in pharmacological approachesfuture of personalized medicineinduced pluripotent stem cells researchinnovative approaches to rare genetic conditionsinnovative therapies for children with genetic disordersmini-organs for rare disease therapyorganoids for drug developmentovercoming bottlenecks in ASO designpatient-specific mutation treatmentpersonalized medicine for childrenrevolutionizing treatment of genetic disordersRNA therapeutics for genetic disordersscalable systems for genetic therapiesscalable systems in genetic researchtargeted therapies for rare diseases
What's Your Reaction?
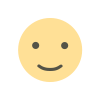
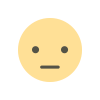
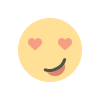
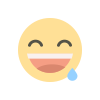
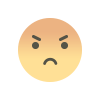
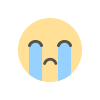
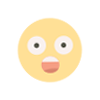